1. Introduction
In recent years, polyvinylidene fluoride (PVDF, (CH2-CF2)n) and its copolymers with trifluoroethylene (P(VDF-TrFE)) have been gaining interest owing to their exciting ferro-, piezo-, and pyroelectric properties. P(VDF-TrFE) thin films have proven to be a promising material for a ferroelectric random access memory because of its low processing temperature [1]. β-phase, among P(VDF-TrFE)’s four crystal phases, exhibits strong ferroelectricity. Since the discovery of strong piezoelectricity of P(VDF) in 1969 [2], PVDF and P(VDF-TrFE) have been extensively studied for their applications in many areas to include energy harvesters [3,4], nonvolatile memories [5], and piezoelectric transducers [6]. On the nanometer scale, P(VDF-TrFE) recently demonstrated interesting behaviors to include highly ordered patterns under strong electric fields [7].
In addition to piezoelectric and related applications, PVDF has been explored for polymer electrolytes [8] of the lithium ion batteries. In this application, polymeric materials such as poly(acrylonitrile) (PAN), poly(methy1 methacrylate) (PMMA), or PVDF are mixed with low-molecular-weight solvents and lithium salt to leverage their mechanical properties and ionic conductivities. This type of polymeric electrolyte is often called a “gel electrolyte” owing to its gel-like viscosity and elasticity. Gel electrolytes are an important component for solid-state or thin film-based batteries and supercapacitors. Gel electrolytes, in solid state and flexible supercapacitors, have attracted a great deal of attention as mechanical durability and stability of flexible energy storage devices inevitably require replacement of conventional liquid electrolytes with polymeric electrolytes.
The electrochemical capacitors or supercapacitors have gained much attention owing to their high power density, long cycle life, and high efficiency compared to both lithium batteries and conventional capacitors [9-11]. Typically, conventional supercapacitors employ liquid electrolytes such as aqueous solutions of H2SO4, KNO3, NaCl, and Na2SO4. Even if these liquid electrolytes generally ensure high ion conductivity, their limited potential window results in a low energy density of the supercapacitors. Furthermore, to avoid the electrolytes, robust encapsulation of liquid-based supercapacitors is required. As a result, the development of gel or polymer electrolytes is inevitable as they have a larger potential window, lower internal corrosion, higher device flexibility, and the capability to avoid potential leakage compared to the conventional liquid electrolytes [12]. In supercapacitor applications of polymeric or gel electrolytes, poly (vinyl alcohol) (PVA) has been widely studied due to its high water absorbing capacity, low cost, and good gel strength [12]. However, the conventional PVA-based electrolytes employ water as a solvent, therefore limiting electrochemical potential window. Since research on polymer electrolytes, for supercapacitors, are still in an early stage, further development of novel polymer gel electrolytes is required and of substantial significance for various applications of the supercapacitors.
While studies on the piezo-, pyro-, and ferroelectric properties and related applications are growing, reports on electrochemical behaviors of PVDF and its copolymers are limited. Due to growing interest in the electrochemical energy storage devices, such as lithium batteries and supercapacitors, there have been numerous electrolytes and electrodes made of polymers.
Herein, we report electrochemical properties of P(VDF-TrFE) dissolved in dimethylformamide (DMF) for its application as an electrolyte of the electrochemical energy storage devices. To our knowledge, this research is some of the first research regarding the P(VDF-TrFE) electrolyte. High density ZnO nanowires were grown in a flexible, conductive fabric to form supercapacitor electrodes. The P(VDF-TrFE) exhibited high specific capacitance up to 18.5 Fg-1. Supercapacitors, using the gel electrolytes, demonstrated high capacitance retention, comparable to the aqueous electrolyte (H2SO4) reported in the literature. Furthermore, the gel electrolyte’s thermal behavior confirmed thermal stability which could be useful for its applications requiring high temperature operations.
The merits of P(VDF-TrFE), as electrolytes for supercapacitors, are mainly three-fold. First, the P(VDF-TrFE) gel electrolytes could provide a larger potential window and thus higher energy density compared to conventional liquid electrolytes. Second, high thermal stability and viscosity of the gel electrolytes enable stable operations of the supercapacitor cells at high temperatures and under mechanical deformation. Third, the electrochemical properties of P(VDF-TrFE), as we found, can be combined with its piezo, pyro, and ferroelectric functionalities to realize multi-functional sensors, energy harvesting, and storage devices.
2. Experimental
The ferroelectric and piezoelectric copolymer powder of P(VDF-TrFE) was purchased from Piezotech. The powder was 70%/30% in mole (VDF/TrFE) composition. The powder was dissolved in DMF (Sigma Aldrich) at a concentration of 20%. Then, the copolymer solution in DMF was used as electrolytes of supercapacitors.
The typical hydrothermal method was used to grow ZnO nanowires (NWs) on flexible and conductive fabrics made of polyethylene terephthalate (PET). First, the PET fabrics were cut into pieces with an area of approximately 1 cm2. The conductive PET fabrics were purchased from Solueta, Korea and coated with a 2-3-μm-thick Au film. Au film on the fabric served as a seed layer to grow ZnO NWs. The fabric pieces were dipped into an aqueous solution of 15 mM of zinc nitrate hexahydrate (98%, Aldrich) and hexamethylenetetramine (99%, Aldrich). The growth solution was heated to 80℃ for 14 h. Two pieces of NWs-grown substrates were employed to form two electrodes of the supercapacitors. The copolymer gel solution of P(VDF-TrFE) in DMF and a glass fiber filter (Whatman) were used as the electrolyte and separator, respectively, for the supercapacitors. All the electrochemical tests were performed using a potentiostat (Versastat 4).
A thermal analysis on the copolymer gel solution was performed using thermogravimetric analyzer (TGA, Q600, TA instruments) with temperature ranging from ambient to 700℃. The electrolyte sample of 1.758 mg was used for this thermogravimetric analysis. To obtain TGA measurements, the samples were heated from room temperature to 700℃ with nitrogen purge gas. The heating rate and nitrogen flow rate were 10℃ min-1 and 100 mL min-1, respectively. Fourier transform infrared spectra were obtained from 700-1400 cm-1 using a FTIR spectrophotometer (Frontier, PerkinElmer). The morphology of the fabric substrates and NWs were investigated by field emission scanning electron microscopy (JEOL) at 15 kV.
3. Results and Discussion
The chemical formula of P(VDF-TrFE) is illustrated in Fig. 1(a). PVDF has monomers of -CH2-CF2-, polyethylene (PE), and polytetrafluoroethylene (PTFE). PVDF is similar to two polymers, PE and PTFE, in the chemical structure resulting in its characteristic chemical stability and physical flexibility [13]. -CF2-CFH- is TrFE. This addition to PVDF causes an increase to the unit cell size. TrFE also modifies inter-planar distance of the ferroelectric phase [13]. TrFe causes a decrease in the interactions between dipole-to-dipole, thereby lowering Curie temperature while the Curie temperature of PVDF is above the melting temperature (195-197℃) [14].
The chemical formula of the solvent, N,N-dimethylformamide (DMF) is also shown in Fig. 1(a). Several solvents, including DMF [15-17], polypropylene carbonate (PC) [8,15], and ethylene carbonate (EC) [18], have been recently reported as solvents for polymer electrolytes of lithium batteries, enhancing conductivity. DMF is an organic solvent capable of being mixed with water and other organic liquids. The high dielectric constant and low viscosity of DMF makes it a suitable solvent of polymer electrolytes for batteries and supercapacitors. Photos of a ZnO NWs-grown flexible PET fabric substrate, used for the supercapacitor and P(VDF-TrFE) dissolved in DMF, are shown in Fig. 1(b).
Fig. 1.
(a) Chemical formula of (a) P(VDF-TrFE) copolymer and N,N-dimethylformamide (DMF). (b) Photo of a ZnO NWs-grown flexible PET substrate used for the supercapacitor cell. The inset: Photo of P(VDF-TrFE) dissolved in DMF.

Fig. 2(a) illustrates the TGA result of the P(VDF-TrFE) gel electrolyte. There was no weight loss of the gel electrolyte up to 400℃, demonstrating its high thermal stability. After 500℃, the weight of the gel electrolyte still retained approximately 91% of its initial weight. Slight weight loss at 150℃ is owed to the low boiling point (150℃) of the solvent, DMF. The thermal stability of P(VDF-TrFE) gel electrolyte is better, or at least comparable, to previously reported organic electrolytes. For example, ionic liquid (IL) N-butyl-N-methylpyrrolidinium bis(trifluorometh-anesulfonyl) imide electrolyte for lithium batteries was reported to show the thermal stability up to 350℃ [19]. The addition of organic carbonate electrolyte, such as propylene carbonate, to this ionic liquid resulted in lower thermal stability [19]. To investigate the interactions between the P(VDF-TrFE) salt and DMF solvent, molecular vibration analysis (Fourier transform infrared spectroscopy (FT-IR)) was employed, analyzing the absorption of infrared light.
FTIR spectra of P(VDF-TrFE) in DMF are shown in Fig. 2(b). Certain characteristic peaks of the P(VDF-TrFE) and DMF were observed in the spectra. It is well known that pure DMF results in a strong peak at 866 cm-1, which is the C-N symmetric stretching mode of vibration. In PVDF mixed with DMF, the band at ~866 cm-1 is transformed into a doublet (~860 cm-1 and ~880 cm-1) [20] which is evident by the FTIR result (Fig. 2(b)). This doublet indicates the interaction between the P(VDF-TrFE) and solvent (DMF). The bands at ~1255 cm-1, 1089 cm-1, and 1063 cm-1 are assigned to be DMF’s characteristic C-N asymmetric vibration and CH3 rocking modes [20]. The bands at ~1386 cm-1 and 844 cm-1 originate from P(VDF-TrFE) [21]. The band at ~1386 cm-1 belongs to the CH2 wagging vibration while the 844 cm-1 band is attributed to the CF2 symmetric stretching of P(VDF-TrFE) [21]. These results indicate that P(VDF-TrFE) was successfully dissolved while interacting with DMF.
Fig. 2.
(a) Thermogravimetric analysis result, and (b) FTIR spectra of P(VDF-TrFE) dissolved in DMF.
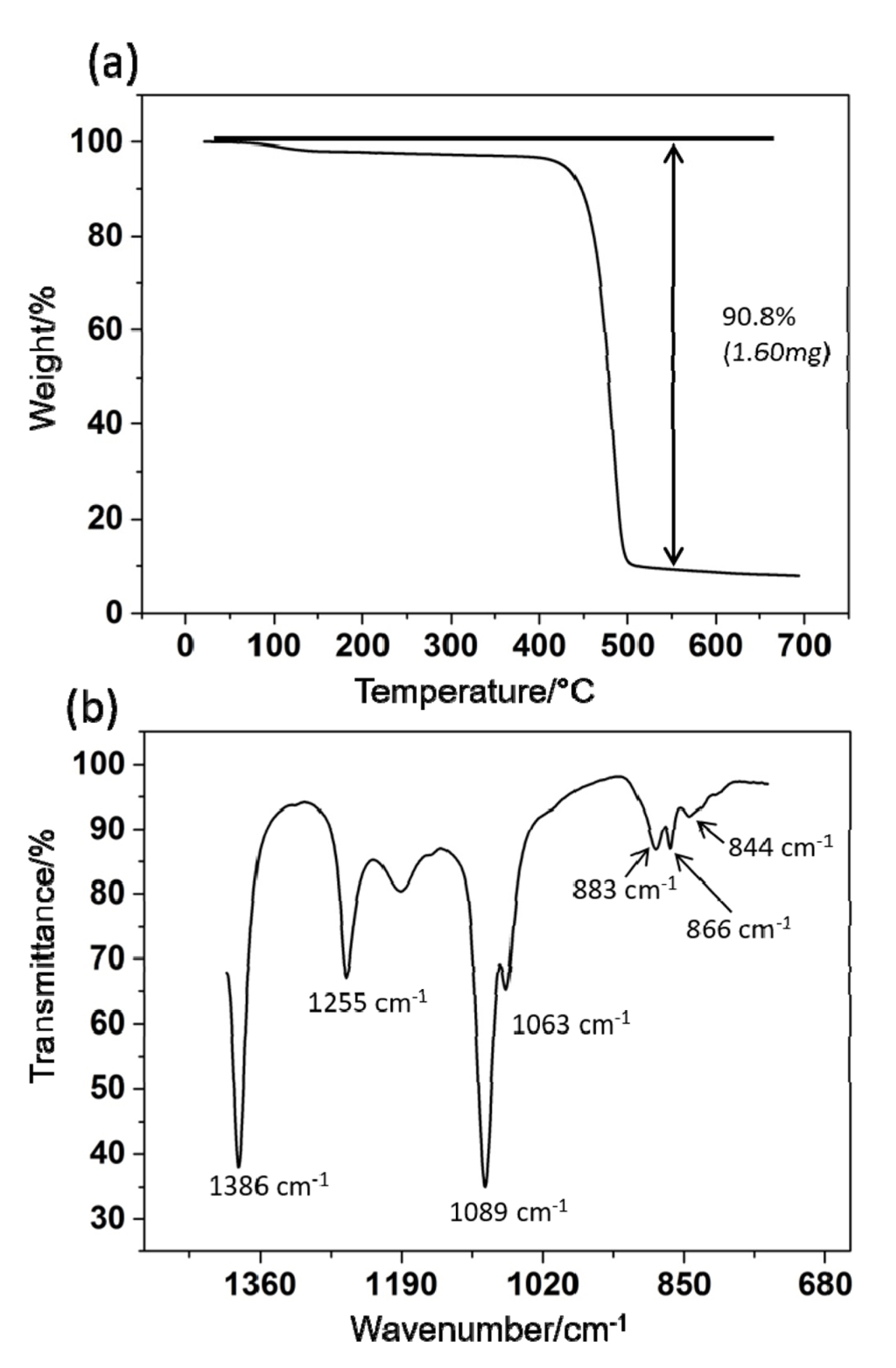
In order to form the supercapacitor electrodes, highly-density ZnO NWs were grown on the flexible PET fabrics as described above. The addition of ZnO NWs to the substrates (PET fabrics) results in a considerable increase to the surface area of the electrodes.
Fig. 3 illustrates scanning electron microscopy (SEM) images of ZnO NWs grown Au-coated PET fabrics. In Fig. 3(a) (low magnification view), the PET fabrics are comprised of interwoven networks of PET fibers. Each fiber has a thickness of approximately 10 μm, as depicted in Fig. 3(b). The curved structures of PET fibers contribute significantly to larger surface areas for the supercapacitor electrodes. The detailed morphology and structure of the nanowires are clearly shown in higher magnification views (Fig. 3(c), (d)). ZnO NWs are densely grown on the substrates. Fig. 3(c) shows typical hexagonal structures of the nanowires. NW’s diameter is about 150 nm.
Fig. 3.
SEM images of ZnO NWs grown on Au-coated PET fabric substrates at different magnifications. (a) Scale bar: 100 μm. The inset: Photo of a ZnO NWs-grown flexible PET substrate. (b) Scale bar: 10 μm. (c) Scale bar: 2 μm. (d) Scale bar: 200 nm.

Fig. 4 demonstrates the electrochemical behaviors of P(VDF-TrFE) gel electrolytes in the supercapacitors using ZnO NWs-grown PET fabrics as electrodes. The cyclic voltammetry (CV) measurements were performed on the supercapacitors using P(VDF-TrFE) gel as electrolytes. The potential range was 0-1 V, and the scan rates were 20, 50, and 100 mVs-1 respectively. The CV curves outlined in Fig. 4(a) reveal the general trend of an increasing current with increasing scan rates. This is a common behavior of aqueous electrolytes for supercapacitors. While the typical aqueous and organic electrolytes exhibit a rectangular shape in CV curves, the shape of the CV curve from the P(VDF-TrFE) gel electrolytes appear more linear than rectangular. However, a clear charge storage process is observed as evidenced by the area of each CV curve and the relationship between the increase in currents and scan rates. As there were no redox peaks on the curves, we conclude that the charge storage occurred by an ideal electric double layer process [22].
The CV curves in Fig. 4(a) are used to determine the mass specific capacitances (Cm) as a function of the potential using Cm = I · S-1 · M-1, where I is the current, S is the scan rate, and M is the mass of the active materials (ZnO NWs) on the electrodes. In Fig. 4(b), the specific capacitances reach up to 18.5 (20 mVs-1), 13.3 (50 mVs-1), and 9.7 Fg-1 (100 mVs-1). The maximum specific capacitances at different scan rates of P(VDF-TrFE) gel electrolytes are shown and compared in Fig. 4(c). The gel electrolytes with ZnO NWs electrodes exhibit the enhanced capacitance for various scan rates. The increased capacitances with decreased scan rates are a typical behavior of supercapacitor electrolytes. At 100 mVs-1, the supercapacitor maintains 52% of its specific capacitance at 20 mVs-1 as illustrated in Fig. 4(d). 52% of capacitance retention of P(VDF-TrFE) gel electrolyte is comparable to 52.9% of 1 M H2SO4 aqueous electrolytes with reduced graphene oxide-SnO2 electrolytes [23]. These results suggest that P(VDF-TrFE) gel possesses increased electrolyte accessibility and electrochemical stability at increased scan rates.
Fig. 4.
(a) Cyclic voltammetry (CV) results from supercapacitors using P(VDF-TrFE) gel electrolytes and ZnO NWs electrodes at the potential range between 0 V to 1 V. The voltage scan rates are 100 mVs-1, 50 mVs-1, and 20 mVs-1. Inset: Schematic showing a supercapacitor cell. (b) Mass specific capacitance vs (c) Mass specific capacitances at the three scan rates. (d) The capacitance retention at the scan rates.
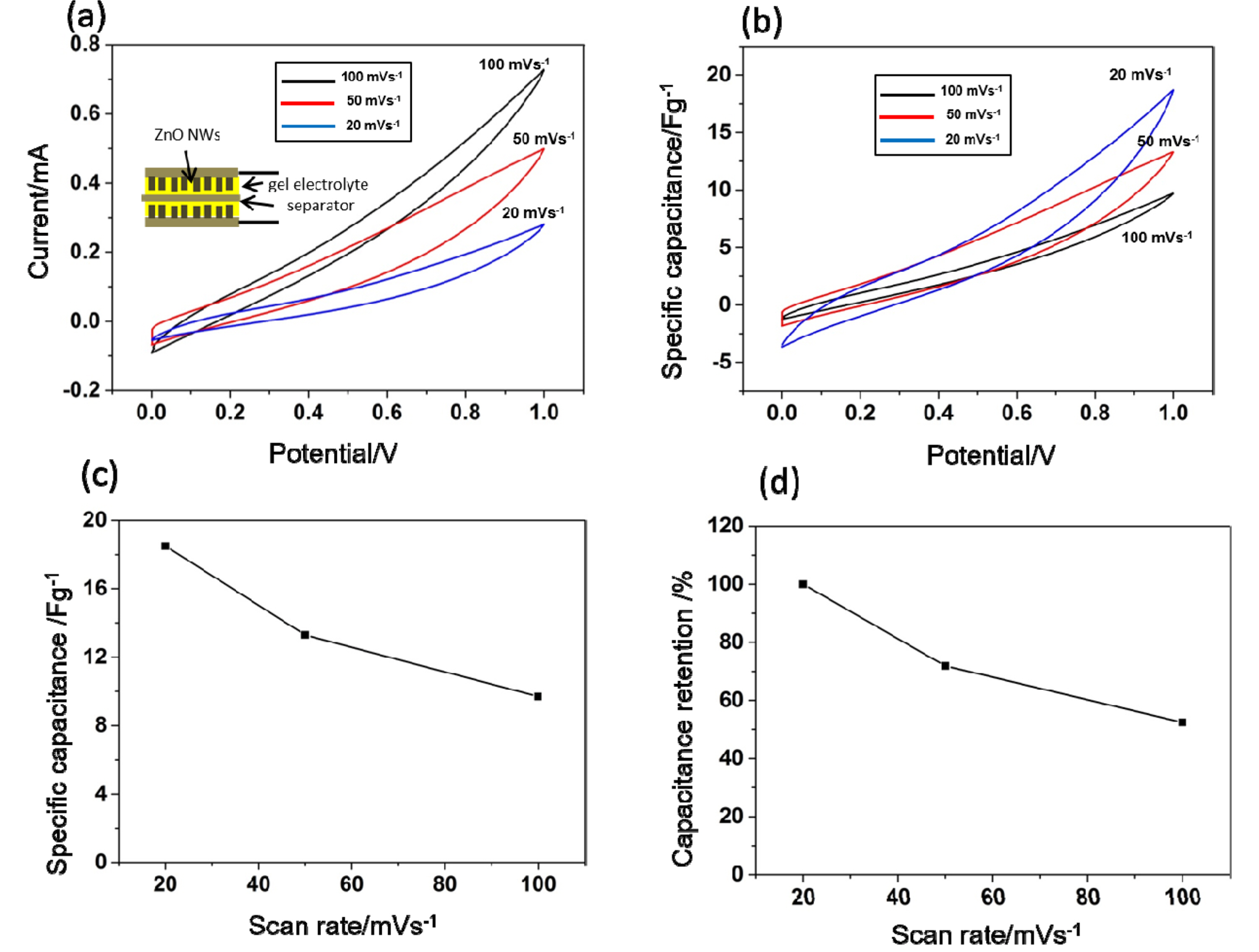
Figure 5(a) shows the galvanostatic charge-discharge curve of P(VDF-TrFE) gel electrolyte at a current of 0.2 mA. The potential range is 0-0.5 V. The curve is linear and symmetrical, suggesting stable, capacitive charging and discharging operations of P(VDF-TrFE) gel electrolyte.
Electrochemical impedance spectroscopy (EIS) measurements were conducted to characterize the AC response and the internal resistance of the supercapacitor. The EIS was carried out at a dc bias of 10 mV (RMS) with a frequency range of 5-800 MHz. The resulting Nyquist graph is presented in Fig. 5(b). From the x-axis intercept, the internal resistance of the supercapacitor, using the gel electrolyte, is 90 Ω.
Fig. 5.
(a) Galvanostatic charge-discharge results from supercapacitors using P(VDF-TrFE) gel electrolytes and ZnO NWs electrodes. (b) The impedance spectrum of the supercapacitor in the 5 MHz to 0.8 Hz frequency range.

To evaluate the mechanical durability and stability of P(VDF-TrFE) gel electrolytes for their application in flexible energy storage devices, the mechanical bending test was conducted on supercapacitors using the gel electrolytes. During the test, the supercapacitor was bent at an angle as illustrated in Fig. 6. The capacitance retention was determined at each bending angle. Fig. 6 shows that the capacitance increases slightly, up to 17%, at a bending angle of 10°. This increase of the capacitance may be attributed to the change in pressure on two electrodes. After 10°, almost constant capacitance was observed as the bending angle increased. This demonstrated the gel electrolyte-based supercapacitor possessed stable performance up to 120°. At 120°, the capacitance retention is 120%. The bending angle and dimensions of PET fabric substrates allow us to determine the strain the supercapacitor experienced. The maximum strain was determined to be approximately 0.53% at 120°, which is comparable to the strains reported from the flexible devices during similar bending tests.
4. Conclusions
In conclusion, we realized all solid-state electro-chemical supercapacitors using novel P(VDF-TrFE) gel electrolytes and ZnO NWs-based electrodes. The specific capacitances, per unit mass of ZnO NWs on the electrodes, are demonstrated be up to 27 Fg-1. These charge-discharge measurements revealed a stable and reliable capacitive response from the supercapacitor cell. The thermal analysis indicated that the polymer electrolytes possess high thermal stability, which is useful for applications in electrochemical energy storage devices operating at high temperatures. All-solid-state, high efficiency electrochemical supercapacitors, using P(VDF-TrFE) electrolytes and ZnO NWs electrodes, demonstrated high specific capacitance and capacitance retention. Furthermore, the gel electrolyte-based supercapacitors demonstrate high mechanical durability up to a bending angle of 120°. Our research demonstrates that P(VDF-TrFE) could be a promising electrolyte for applications in flexible and fabric-based energy storage devices.